This article first appeared in the Autumn 2012 issue of Energy Futures, the magazine of the MIT Energy Initiative. Subscribe today.
Much attention is now focusing on natural organisms that have evolved highly efficient light-harvesting capabilities over billions of years. The green sulfur bacterium is perhaps the champion light-harvester. It lives at the depths of the ocean, where light levels are extremely low. But it makes the most of the light it gets: It is able to harvest up to 95% of the solar energy it absorbs.
Research teams worldwide are trying to replicate the capabilities of the green sulfur bacterium. But such natural systems are exceedingly complex. “We’d like to break these systems down into their simpler components and see if we can find synthetic [structures] that mimic them,” says Moungi G. Bawendi, the Lester Wolfe Professor of Chemistry. “In the end, we want a set of building blocks that we can assemble to create a more complicated system — perhaps as complex as the natural system but where we have more control, where we can tune some critical parameters.”
Key to the light-harvesting success of the green sulfur bacterium is its light-harvesting antenna system. This system consists of long cylinders of closely packed bacteriochlorophyll molecules that absorb solar energy and transfer as much as 95% of it toward the “reaction centers” where critical chemical processes occur. Those cylinders are “perhaps nature’s most spectacular light-harvesting system,” says Dörthe M. Eisele, postdoctoral associate in the MIT Research Laboratory of Electronics. They could be an ideal building block for a practical device.
But applications are far down the road. The first step is to develop a fundamental understanding of how nature’s bacteriochlorophyll cylinders do their job. Chopping individual cylinders out of a bacterium for analysis would destroy their natural functioning. The solution is therefore to create and study an artificial model system that behaves like the in situ bacterio-chlorophyll cylinders.
A model system — and a challenge
For the past five years, Eisele and her collaborators at MIT’s Center for Excitonics, the University of Texas at Austin, Humboldt University of Berlin in Germany and the University of Groningen in the Netherlands have been working with an artificial system that is similar in size, shape, and function to the natural antenna system in the green sulfur bacterium.
The structure consists of molecules of cyanine dye that naturally aggregate and self-assemble, rolling up into long double-walled nanotubes when they are immersed in water. Each nanotube is about 13 nm wide and thousands of times that long, and each contains two concentric cylinders of closely packed cyanine dye molecules about 4 nm apart. That “supramolecule” with its two cylinders of light-absorbing material closely resembles the natural antenna system in the green sulfur bacterium.
In 2007, Eisele developed a carefully controlled technique to produce well-defined cyanine dye-based nanotubes. In early work, she and her colleagues demonstrated that her nanotubes have highly uniform properties — from tube to tube and also along the length of each one. Because the LH nanotubes are “all the same,” she can study their properties from the ensemble in solution with no need to isolate the responses of the individual nanotubes.
Nevertheless, determining how the LH nanotubes collect and transport energy from light is a challenge. Even advanced microscopes cannot show the details of their structure, so Eisele turned to another option: their optical spectra. Shine light on a supramolecule made up of closely packed molecules and it will absorb certain wavelengths and not others. The resulting spectrum can be the key to unraveling not only the optical behavior of the supramolecule but also its physical structure.
Early spectral analyses revealed a critical property of such cyanine-based supramolecules. On their own, the individual cyanine molecules have a characteristic absorption spectrum. Yet when they pack together, the supramolecule that results has a dramatically different spectrum — even though the molecules retain their individual structure, do not share electrons, and are held together only by weak attractive forces.
Why? When an individual molecule absorbs energy from the sun, it becomes “excited.” But when a supramolecule absorbs solar energy, the closely packed molecules in it “share” their excited states. Because of those shared excited states, the optical properties of the supramolecule are significantly different from those of the individual molecules. “So the unique ability of our nanotubes to harvest light so efficiently arises from the ensemble of closely packed, aggregated molecules,” says Eisele. Moreover, the details of how the molecules are packed together strongly affect those interactions, and thus the optical properties of the supramolecule. If the researchers could understand the relationship between the structural details and the optical properties, they might be able to fine-tune the optical behavior by altering how the molecules pack together.
Understanding the spectral evidence
Analyzing the spectrum of the supra-molecule would provide valuable insights — but there is a problem. The “electron excitation” is shared between neighboring molecules. But do such molecule-to-molecule interactions occur mainly within the inner and outer cylinders separately or throughout the entire LH nanotube? If the former, then the spectrum of the LH nanotube would show the absorption behavior of the two independent (or at most weakly interacting) cylinders superimposed on one another. But if the latter, the overall spectrum would reflect the combined optical response of the two strongly interacting cylinders.
A solution would be to observe the spectra of the cylinders separately. But using a beam of light to excite selected parts of the LH nanotubes in a vial isn’t feasible, and removing the outer cylinder to isolate the spectrum of the inner cylinder won’t work. “It’s a self-assembling system, and we can’t destroy its structure without altering its behavior,” says Eisele. “What we can do is change the light-absorption capability of the outer cylinder.”
To do that, she oxidizes the nanotubes using silver nitrate. Each silver ion removes one electron from a single molecule of cyanine dye, altering its absorption behavior. Her experimental procedure therefore consisted of simply adding silver nitrate to a vial of suspended nanotubes and then carefully taking the absorption spectrum of the mixture every 30 minutes for six hours as oxidation proceeded. The results appear in the diagram below.
Experimental results from oxidizing the cyanine-based nanotubes with silver nitrate (AgNO3). The plots show the absorption spectrum of the nanotubes in solution before the AgNO3 was added (red), spectra taken every 30 minutes after it was added (gray), and the spectrum at the end of the six-hour experiment (green). Peak 2 drops down quickly and finally disappears. Peak 1 and other portions of the spectrum change more slowly and drop only in amplitude, not shape. Analysis of the spectral data shows that those two responses reflect the separate behavior of the inner and outer cylinders in the light-harvesting nanotubes. Micrographs show that the outer cylinder is morphologically intact after oxidation but now 'decorated' with silver nanoparticles (as shown on the inset above). Isolation of the inner cylinder’s spectrum subsequently enabled the researchers to model the detailed supramolecular structure of the artificial nanotubes.
The red curve is the initial absorption spectrum before the start of the experiment; the gray curves are the 30-minute spectra; and the green curve is the final spectrum. Comparison of the curves shows that peak 2 goes down more quickly than peak 1 does — and it ultimately disappears. Eisele and her co-workers were able to show that this fast decrease in intensity of peak 2 reflects changes in the outer cylinder and that the slower decrease of peak 1 reflects changes in the inner cylinder. The conclusion: Peak 2 can be unambiguously attributed to the outer cylinder, peak 1 to the inner one. Moreover, the portions of the absorption spectrum that drop only in amplitude with no significant change in shape can be traced to the inner cylinder.
Detailed analysis of the experimental data confirmed that the original spectrum is made up of the spectra of two essentially independent chemical species superimposed on one another. The two LH cylinders can thus be treated as two electronically separate systems, with at most weak coupling between them. In addition, images taken with a cryogenic electron transmission microscope clearly showed the double-walled structure of the nanotubes — both before and after oxidation. Indeed, the only difference in the post-oxidation images was that the exterior surfaces of the nanotubes were “decorated with silver nanoparticles,” says Eisele. Those results confirm that the outer cylinder was still physically present. Only its optical behavior had changed.
The isolation of the inner cylinder’s spectrum made possible unprecedented theoretical advances. After three years’ work, collaborators led by Professor Jasper Knoester at the University of Groningen, the Netherlands, modeled a structure for the inner cylinder that reproduced the experimentally observed spectrum. The structure has molecules organized in a herring-bone fashion — a geometry previously proposed by others but with certain details that are different. For example, each tile is made up of two molecules, and as the tiles wrap around to form the cylinder, they tilt out from the surface at distinctive angles.
Knoester and his group next modeled the structure of the outer cylinder assuming the same packing geometry but adjusted to span the greater circumference. They then calculated the spectrum of a suprastructure formed from their two cylinders that shows remarkable agreement with Eisele’s measured absorption spectrum. By combining these experimental and theoretical results, the researchers were thus able to settle a long-standing argument about the geometry of such cyanine-based nanotubes.
Only the beginning
Armed with their new understanding, the researchers at MIT are now continuing their studies. For example, they are examining the nature of the weak interaction between the inner and outer cylinders, and they are looking into what happens when many cylindrical nanotubes cluster together, as they do in nature. Says Eisele, “Now we need to know whether we can think of them as a superposition of individual cylinders — or do they become a totally new system with different optical properties?”
But even a cluster of LH cylinders is just one building block for a future device, Bawendi says. He and Eisele are now working to connect the LH nanotubes to quantum dots (QDs) — nanometer-scale inorganic crystals that fluoresce when stimulated by light. That combination raises exciting possibilities. By controlling the size of the QDs, Bawendi — an expert in this field — will be able to “tune” them to absorb sunlight and then emit a specific wavelength that will generate maximum electron excitation in the LH nanotubes. In the lab, that focused light will enable the researchers to track how the excitation propagates along the LH nanotubes. In a practical device, such tailored QDs could deliver focused energy that LH nanotubes could efficiently transport and deliver to a system — perhaps including more QDs — where chemical reactions might, for instance, produce fuels.
Bawendi stresses that such concepts are very far down the line. “The idea is to create something from building blocks, so first we have to understand the building blocks themselves and how they interact,” he says. But if his “grand vision” succeeds, a device integrating such building blocks could one day provide a completely new way to collect energy from the sun — perhaps modeled in part on that solar-harvesting genius, the green sulfur bacterium.
This research was supported by the MIT Center for Excitonics, an Energy Frontier Research Center funded by the US Department of Energy, Office of Science, Office of Basic Energy Sciences, and by the Deutsche Forschungsgemeinschaft, the Integrative Research Institute for the Sciences in Berlin, the National Science Foundation, the Alexander von Humboldt Foundation, the US Army Research Office and the US Defense Advanced Research Projects Agency.
Further information can be found in:
D.M. Eisele, C.W. Cone, E.A. Bloemsma, S.M. Vlaming, C.G.F. van der Kwaak, R.J. Silbey, M.G. Bawendi, J. Knoester, J.P. Rabe, and D.A. Vanden Bout. “Utilizing redox-chemistry to elucidate the nature of exciton transitions in supramolecular dye nanotubes.” Nature Chemistry, vol. 4, pp. 655–662, July 2012.
B.J. Walker, V. Bulović, and M.G. Bawendi. “Quantum dot/J-aggregate blended films for light harvesting and energy transfer.” Nano Letters, vol. 10, pp. 3995–3999, 2010.
D.M. Eisele, J. Knoester, S. Kirstein, J.P. Rabe, and D.A. Vanden Bout. “Uniform exciton fluorescence from individual molecular nanotubes immobilized on solid substrates.” Nature Nanotech, vol. 4, pp. 658–663, 2009.
B.J. Walker, G.P. Nair, L.F. Marshall, V. Bulović, and M.G. Bawendi. “Narrow-band absorption-enhanced quantum dot/J-aggregate conjugates.” Journal of the American Chemical Society, vol. 31, pp. 9624–9625, 2009.
Much attention is now focusing on natural organisms that have evolved highly efficient light-harvesting capabilities over billions of years. The green sulfur bacterium is perhaps the champion light-harvester. It lives at the depths of the ocean, where light levels are extremely low. But it makes the most of the light it gets: It is able to harvest up to 95% of the solar energy it absorbs.
Research teams worldwide are trying to replicate the capabilities of the green sulfur bacterium. But such natural systems are exceedingly complex. “We’d like to break these systems down into their simpler components and see if we can find synthetic [structures] that mimic them,” says Moungi G. Bawendi, the Lester Wolfe Professor of Chemistry. “In the end, we want a set of building blocks that we can assemble to create a more complicated system — perhaps as complex as the natural system but where we have more control, where we can tune some critical parameters.”
Key to the light-harvesting success of the green sulfur bacterium is its light-harvesting antenna system. This system consists of long cylinders of closely packed bacteriochlorophyll molecules that absorb solar energy and transfer as much as 95% of it toward the “reaction centers” where critical chemical processes occur. Those cylinders are “perhaps nature’s most spectacular light-harvesting system,” says Dörthe M. Eisele, postdoctoral associate in the MIT Research Laboratory of Electronics. They could be an ideal building block for a practical device.
But applications are far down the road. The first step is to develop a fundamental understanding of how nature’s bacteriochlorophyll cylinders do their job. Chopping individual cylinders out of a bacterium for analysis would destroy their natural functioning. The solution is therefore to create and study an artificial model system that behaves like the in situ bacterio-chlorophyll cylinders.
A model system — and a challenge
For the past five years, Eisele and her collaborators at MIT’s Center for Excitonics, the University of Texas at Austin, Humboldt University of Berlin in Germany and the University of Groningen in the Netherlands have been working with an artificial system that is similar in size, shape, and function to the natural antenna system in the green sulfur bacterium.
The structure consists of molecules of cyanine dye that naturally aggregate and self-assemble, rolling up into long double-walled nanotubes when they are immersed in water. Each nanotube is about 13 nm wide and thousands of times that long, and each contains two concentric cylinders of closely packed cyanine dye molecules about 4 nm apart. That “supramolecule” with its two cylinders of light-absorbing material closely resembles the natural antenna system in the green sulfur bacterium.
In 2007, Eisele developed a carefully controlled technique to produce well-defined cyanine dye-based nanotubes. In early work, she and her colleagues demonstrated that her nanotubes have highly uniform properties — from tube to tube and also along the length of each one. Because the LH nanotubes are “all the same,” she can study their properties from the ensemble in solution with no need to isolate the responses of the individual nanotubes.
Nevertheless, determining how the LH nanotubes collect and transport energy from light is a challenge. Even advanced microscopes cannot show the details of their structure, so Eisele turned to another option: their optical spectra. Shine light on a supramolecule made up of closely packed molecules and it will absorb certain wavelengths and not others. The resulting spectrum can be the key to unraveling not only the optical behavior of the supramolecule but also its physical structure.
Early spectral analyses revealed a critical property of such cyanine-based supramolecules. On their own, the individual cyanine molecules have a characteristic absorption spectrum. Yet when they pack together, the supramolecule that results has a dramatically different spectrum — even though the molecules retain their individual structure, do not share electrons, and are held together only by weak attractive forces.
Why? When an individual molecule absorbs energy from the sun, it becomes “excited.” But when a supramolecule absorbs solar energy, the closely packed molecules in it “share” their excited states. Because of those shared excited states, the optical properties of the supramolecule are significantly different from those of the individual molecules. “So the unique ability of our nanotubes to harvest light so efficiently arises from the ensemble of closely packed, aggregated molecules,” says Eisele. Moreover, the details of how the molecules are packed together strongly affect those interactions, and thus the optical properties of the supramolecule. If the researchers could understand the relationship between the structural details and the optical properties, they might be able to fine-tune the optical behavior by altering how the molecules pack together.
Understanding the spectral evidence
Analyzing the spectrum of the supra-molecule would provide valuable insights — but there is a problem. The “electron excitation” is shared between neighboring molecules. But do such molecule-to-molecule interactions occur mainly within the inner and outer cylinders separately or throughout the entire LH nanotube? If the former, then the spectrum of the LH nanotube would show the absorption behavior of the two independent (or at most weakly interacting) cylinders superimposed on one another. But if the latter, the overall spectrum would reflect the combined optical response of the two strongly interacting cylinders.
A solution would be to observe the spectra of the cylinders separately. But using a beam of light to excite selected parts of the LH nanotubes in a vial isn’t feasible, and removing the outer cylinder to isolate the spectrum of the inner cylinder won’t work. “It’s a self-assembling system, and we can’t destroy its structure without altering its behavior,” says Eisele. “What we can do is change the light-absorption capability of the outer cylinder.”
To do that, she oxidizes the nanotubes using silver nitrate. Each silver ion removes one electron from a single molecule of cyanine dye, altering its absorption behavior. Her experimental procedure therefore consisted of simply adding silver nitrate to a vial of suspended nanotubes and then carefully taking the absorption spectrum of the mixture every 30 minutes for six hours as oxidation proceeded. The results appear in the diagram below.
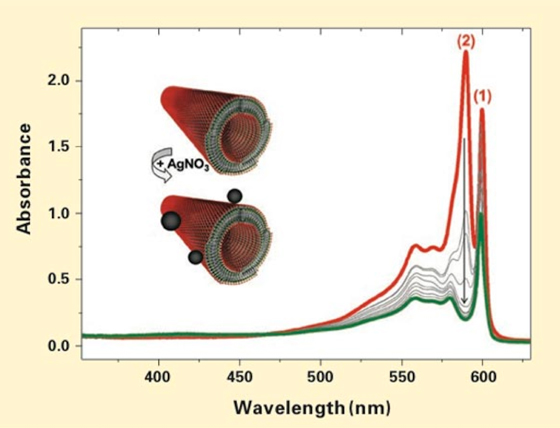
Experimental results from oxidizing the cyanine-based nanotubes with silver nitrate (AgNO3). The plots show the absorption spectrum of the nanotubes in solution before the AgNO3 was added (red), spectra taken every 30 minutes after it was added (gray), and the spectrum at the end of the six-hour experiment (green). Peak 2 drops down quickly and finally disappears. Peak 1 and other portions of the spectrum change more slowly and drop only in amplitude, not shape. Analysis of the spectral data shows that those two responses reflect the separate behavior of the inner and outer cylinders in the light-harvesting nanotubes. Micrographs show that the outer cylinder is morphologically intact after oxidation but now 'decorated' with silver nanoparticles (as shown on the inset above). Isolation of the inner cylinder’s spectrum subsequently enabled the researchers to model the detailed supramolecular structure of the artificial nanotubes.
Detailed analysis of the experimental data confirmed that the original spectrum is made up of the spectra of two essentially independent chemical species superimposed on one another. The two LH cylinders can thus be treated as two electronically separate systems, with at most weak coupling between them. In addition, images taken with a cryogenic electron transmission microscope clearly showed the double-walled structure of the nanotubes — both before and after oxidation. Indeed, the only difference in the post-oxidation images was that the exterior surfaces of the nanotubes were “decorated with silver nanoparticles,” says Eisele. Those results confirm that the outer cylinder was still physically present. Only its optical behavior had changed.
The isolation of the inner cylinder’s spectrum made possible unprecedented theoretical advances. After three years’ work, collaborators led by Professor Jasper Knoester at the University of Groningen, the Netherlands, modeled a structure for the inner cylinder that reproduced the experimentally observed spectrum. The structure has molecules organized in a herring-bone fashion — a geometry previously proposed by others but with certain details that are different. For example, each tile is made up of two molecules, and as the tiles wrap around to form the cylinder, they tilt out from the surface at distinctive angles.
Knoester and his group next modeled the structure of the outer cylinder assuming the same packing geometry but adjusted to span the greater circumference. They then calculated the spectrum of a suprastructure formed from their two cylinders that shows remarkable agreement with Eisele’s measured absorption spectrum. By combining these experimental and theoretical results, the researchers were thus able to settle a long-standing argument about the geometry of such cyanine-based nanotubes.
Only the beginning
Armed with their new understanding, the researchers at MIT are now continuing their studies. For example, they are examining the nature of the weak interaction between the inner and outer cylinders, and they are looking into what happens when many cylindrical nanotubes cluster together, as they do in nature. Says Eisele, “Now we need to know whether we can think of them as a superposition of individual cylinders — or do they become a totally new system with different optical properties?”
But even a cluster of LH cylinders is just one building block for a future device, Bawendi says. He and Eisele are now working to connect the LH nanotubes to quantum dots (QDs) — nanometer-scale inorganic crystals that fluoresce when stimulated by light. That combination raises exciting possibilities. By controlling the size of the QDs, Bawendi — an expert in this field — will be able to “tune” them to absorb sunlight and then emit a specific wavelength that will generate maximum electron excitation in the LH nanotubes. In the lab, that focused light will enable the researchers to track how the excitation propagates along the LH nanotubes. In a practical device, such tailored QDs could deliver focused energy that LH nanotubes could efficiently transport and deliver to a system — perhaps including more QDs — where chemical reactions might, for instance, produce fuels.
Bawendi stresses that such concepts are very far down the line. “The idea is to create something from building blocks, so first we have to understand the building blocks themselves and how they interact,” he says. But if his “grand vision” succeeds, a device integrating such building blocks could one day provide a completely new way to collect energy from the sun — perhaps modeled in part on that solar-harvesting genius, the green sulfur bacterium.
This research was supported by the MIT Center for Excitonics, an Energy Frontier Research Center funded by the US Department of Energy, Office of Science, Office of Basic Energy Sciences, and by the Deutsche Forschungsgemeinschaft, the Integrative Research Institute for the Sciences in Berlin, the National Science Foundation, the Alexander von Humboldt Foundation, the US Army Research Office and the US Defense Advanced Research Projects Agency.
Further information can be found in:
D.M. Eisele, C.W. Cone, E.A. Bloemsma, S.M. Vlaming, C.G.F. van der Kwaak, R.J. Silbey, M.G. Bawendi, J. Knoester, J.P. Rabe, and D.A. Vanden Bout. “Utilizing redox-chemistry to elucidate the nature of exciton transitions in supramolecular dye nanotubes.” Nature Chemistry, vol. 4, pp. 655–662, July 2012.
B.J. Walker, V. Bulović, and M.G. Bawendi. “Quantum dot/J-aggregate blended films for light harvesting and energy transfer.” Nano Letters, vol. 10, pp. 3995–3999, 2010.
D.M. Eisele, J. Knoester, S. Kirstein, J.P. Rabe, and D.A. Vanden Bout. “Uniform exciton fluorescence from individual molecular nanotubes immobilized on solid substrates.” Nature Nanotech, vol. 4, pp. 658–663, 2009.
B.J. Walker, G.P. Nair, L.F. Marshall, V. Bulović, and M.G. Bawendi. “Narrow-band absorption-enhanced quantum dot/J-aggregate conjugates.” Journal of the American Chemical Society, vol. 31, pp. 9624–9625, 2009.